For the Instructor
These student materials complement the Modeling Earth Systems Instructor Materials. If you would like your students to have access to the student materials, we suggest you either point them at the Student Version which omits the framing pages with information designed for faculty (and this box). Or you can download these pages in several formats that you can include in your course website or local Learning Managment System. Learn more about using, modifying, and sharing InTeGrate teaching materials.Unit 6 Reading: Great Basin Lakes — Nature's Rain Gauges
by Kirsten Menking, Vassar College
In this week's modeling project, we explore how geologists use clues in the landscape to uncover Earth's history of climatic change. We take, as our example, the Owens River chain of lakes in eastern California, which repeatedly overflowed during the Pleistocene, leaving behind evidence of water balance fluctuations in ancient shorelines and basin center deposits. We consider what climatic conditions may have been necessary to create lakes at different levels and run experiments to determine the ability of the lake chain to record climatic oscillations of different cycle lengths.
Great Basin "Pluvial" Lakes
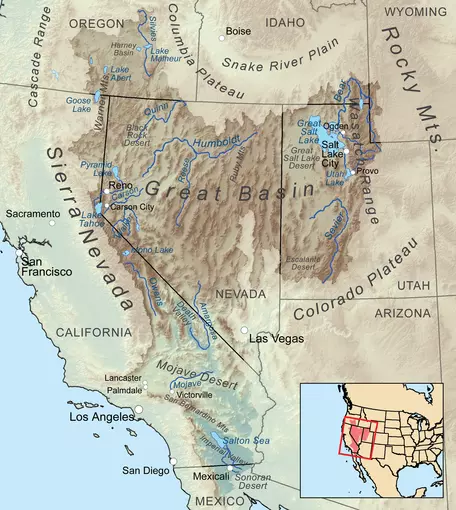
![[creative commons]](/images/creativecommons_16.png)
The Great Basin of the western United States stretches from eastern Oregon southward to southeastern California, eastward into Utah, and underlies nearly all of the state of Nevada (Fig. 1). It is a region formed by stretching of Earth's crust and consists of north-south trending fault-bounded mountain ranges separated by tectonically down-dropped valleys, a landscape once described by geologist G.K. Gilbert (1875) as "an army of caterpillars marching north out of Mexico." Hydrologically, the Great Basin has no connection to any of the large river systems that drain the North American continent. As a result, precipitation that falls within its boundaries has no way to leave except by evaporation.
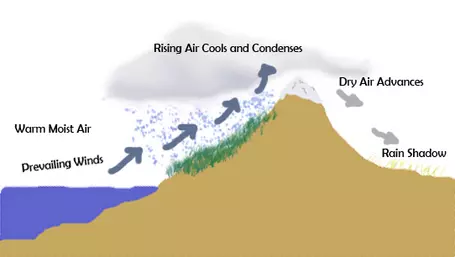
![[reuse info]](/images/information_16.png)
Climatologically, the region is under the influence of storm systems that originate over the Pacific Ocean and work their way eastward on the prevailing westerly winds. As these storm systems cross the Sierra Nevada range in California, the air is forced to rise, causing it to cool and the water vapor it contains to condense to rain and snow that falls on the mountains (Fig. 2). Wrung of most of its moisture, the air continues eastward and loses still more moisture with each mountain range it crosses. For this reason, the interior of the Great Basin is quite arid. Large lakes fed by spring snowmelt exist in a few places, but most valley bottoms instead contain playas (Fig. 3), vast expanses of clay and/or evaporite minerals seasonally flooded by shallow sheets of water that evaporate within a few days to weeks.
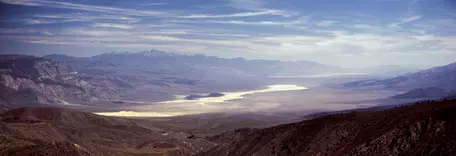
![[reuse info]](/images/information_16.png)
During the Pleistocene, conditions were quite different. Wave-cut shorelines (Fig. 4), beach gravels, and aquatic fossils found high above presently dry valley floors prove that large lakes were the rule rather than the exception at various times in the past. Indeed, the valleys of the Great Basin have been named "nature's rain gauges" (Smith and Bischoff, 1997) since they filled up with water during wet climate intervals and dried up during more arid periods. Lakes that fluctuate in size as climate changes are also called "pluvial" lakes from the Latin word pluvia, meaning rain.
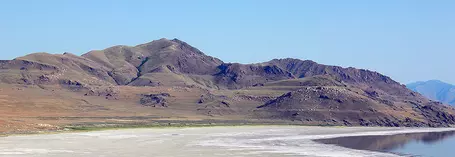
![[reuse info]](/images/information_16.png)
The most extensive of the Pleistocene pluvial lakes was Lake Bonneville, which covered much of western Utah during the last glacial maximum and was 10 times larger than its modern remnant, Great Salt Lake (Fig. 5). Another massive water body, Lake Lahontan, covered the northwestern corner of the state of Nevada. Its remnants include Pyramid and Walker Lakes along with playas known as the Carson Sink, Humboldt Sink, and Winnemucca Lake. Both Bonneville and Lahontan grew to incorporate multiple Great Basin valleys, but many other valleys were occupied by single lakes at the same time.
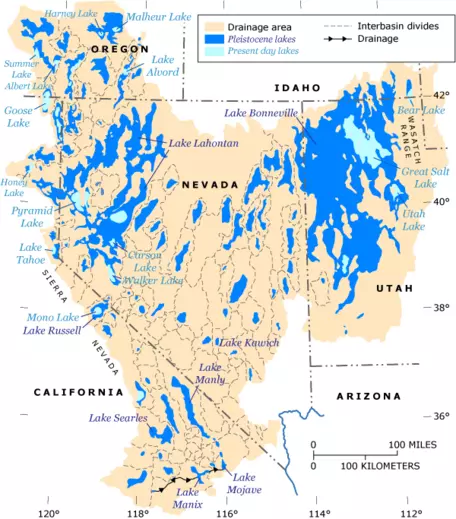
![[reuse info]](/images/information_16.png)
What caused lakes throughout the Great Basin to expand during the Pleistocene? The answer likely has to do with changes in atmospheric circulation. The polar jet stream is a fast-moving current of air that lies at the confluence of the atmospheric polar and ferrel cells where air is rising upward from Earth's surface (Fig. 6). As the air rises, it cools, and the water vapor it contains condenses to rain drops or snow flakes. As such, the polar jet stream is a locus of storm activity.
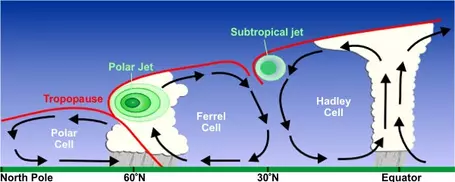
![[reuse info]](/images/information_16.png)
The position of the polar jet stream meanders quite a bit on a week-by-week basis, but today it generally enters the North American continent from the Pacific Ocean somewhere between 50 and 60° latitude, or about the location of British Columbia, Canada. Climate modeling results (Kutzbach, 1987) suggest that growth of the ice sheet that covered the northern half of North America at the last glacial maximum may have deflected the polar jet stream southward (Fig. 7).
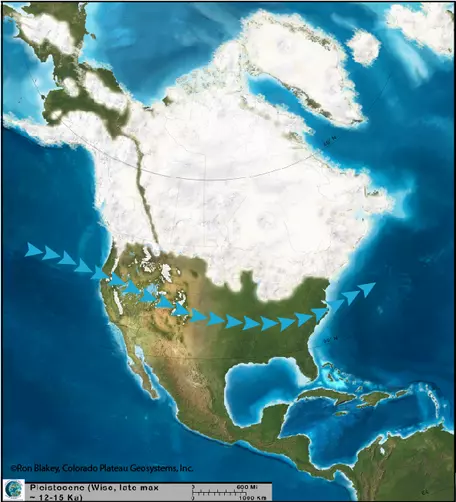
![[reuse info]](/images/information_16.png)
Thus deflected, the locus of storm activity would also have shifted, bringing higher rainfall rates to the desert West. When combined with cooler temperatures from the glaciation and associated lower evaporation rates, these conditions could have promoted the growth of exceptionally large lakes. We know from evidence in ocean sediment cores that periods of glaciation have occurred repeatedly throughout the Pleistocene. If model results for the last glacial maximum are correct, then it is likely that southward deflection of the jet stream occurred on numerous occasions.
The Owens River chain of lakes

![[creative commons]](/images/creativecommons_16.png)
In eastern California, southward migration of the jet stream during global glaciations led to increased snowfall and expansion of glaciers in the Sierra Nevada range. Glacial melt water flowed down dozens of tributaries into the Owens River, where it fed a chain of lakes separated by bedrock sills (Fig. 8). This lake chain may, on occasion, have included Lake Russell, a greatly expanded version of present day Mono Lake in the Mono Basin just north of the Owens Valley, but generally started with the Owens River, which flows into Owens Lake. When Owens Lake filled to its drainage divide, it overflowed into the China Lake basin, which subsequently overflowed into the Searles Lake basin. During exceptionally wet periods, China and Searles Lakes coalesced, filled to a common sill level, and spilled into Panamint Valley and ultimately into Death Valley (Smith and Street-Perrott, 1983; Phillips, 2008). Surface area for the entire chain of lakes exceeded nine times the modern value at such times.
The timing of different overflow events has been pieced together using both shoreline and sediment core evidence from all of the lake basins, and it appears that for much of the Pleistocene, Searles Lake was at the end of the lake chain (Phillips, 2008). The reason we know this is that the Searles valley contains thick deposits of fairly soluble minerals that could only have come from the evaporation of vast quantities of lake water. The precipitated minerals include trona (Na3(CO3)(HCO3)-2H2O), which is used in the manufacture of glass, and borax (Na2[B4O5(OH)4]·8H2O), which is used as an ingredient in laundry detergent; they and many others have been mined from the Searles lake bed for decades. Had Searles Lake overflowed to Panamint Valley most of the time, the dissolved ions that precipitated to form these minerals would have been transported farther down the lake chain, and Searles Lake would instead have filled with clastic sediments (silts and clays) delivered from upstream. Likewise, had Owens Lake been the terminus of the chain, we would expect to find these evaporites in the Owens Valley. A sediment core taken from Owens Lake in the early 1990s shows no evidence of such minerals prior to the present day, indicating that Owens Lake must have overflowed throughout most of the Pleistocene (Smith et al., 1997; Phillips, 2008). Based on the collective evidence from all of the lake basins, it appears that Owens River water flowed all the way to Death Valley around 150,000 years ago and possibly again 70,000 years ago, and that either Searles or Panamint Valley served as the terminal lake basin much of the rest of the time. Owens Lake only rarely dropped below its spillway level and probably served as the terminus of the lake chain for only a few thousand years at a time before receiving sufficient runoff to again overflow.
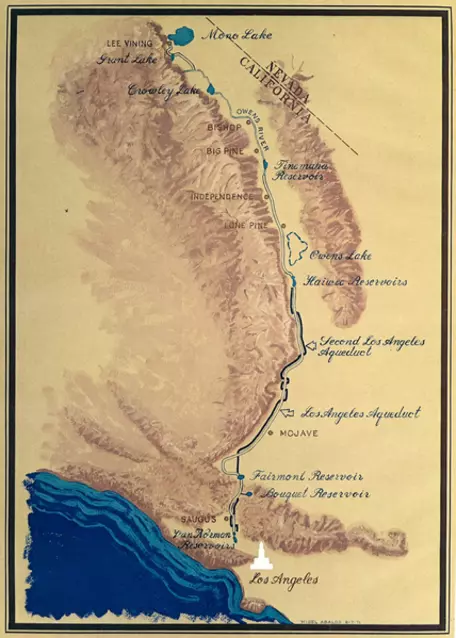
![[reuse info]](/images/information_16.png)
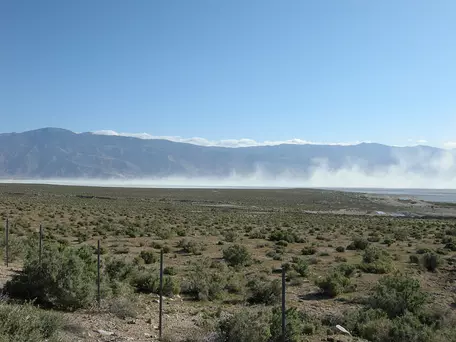
![[creative commons]](/images/creativecommons_16.png)
Today, all of the lakes but Owens and Mono are dried up, the desiccation occurring as the jet stream shifted back to the north at the end of the last glaciation. In addition to responding to this natural climatic change, Owens and Mono also fell victim to the thirsty Los Angeles basin, which exports Owens Valley and Mono Basin water 200 km to the south to supply millions of people with drinking water (Fig. 9). At the turn of the 20th century, Owens Lake was 14 m deep and supported a thriving waterfowl population. After Los Angeles Department of Water and Power engineer William Mulholland built the Los Angeles aqueduct that intercepts the flow of Owens River tributaries, the lake disappeared, leaving behind a dry salt flat whose sediments were blown into dust storms for decades (swhydro.arizona.edu/archive/V3_N4/feature4.pdf, Fig. 10). As Owens Valley residents began to suffer from some of the worst air quality in the nation, a series of court orders issued in the 1990s required the city to return some water to the dried-up lake bed and to implement additional dust mitigation strategies, such as the planting of salt-tolerant vegetation that would protect the surface from the scouring effects of the wind.

![[creative commons]](/images/creativecommons_16.png)
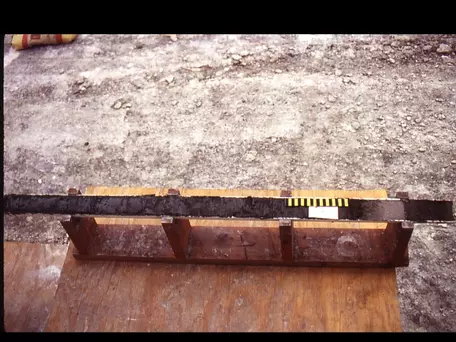
![[creative commons]](/images/creativecommons_16.png)
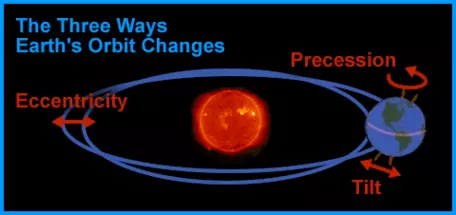
![[creative commons]](/images/creativecommons_16.png)
Hydrologic balance of a lake
While ancient shorelines and basin center deposits of Great Basin pluvial lakes provide evidence that climate must have been much wetter during glaciations, it would be nice to be more quantitative and to determine just how much wetter conditions must have been to create lakes of various sizes. To answer this question, it is helpful to create a model that keeps track of the various inflows to and outflows from each lake and that incorporates information on each basin's topographic characteristics so that we can determine when a particular lake has reached the overflow point. We can then experiment with various combinations of runoff and evaporation to see which conditions could have led to the lake levels we know existed at various times in the past.

![[reuse info]](/images/information_16.png)
In constructing our model of the Owens River chain of lakes, we use the following equation to keep track of the water balance within each individual lake (Fig. 14):
` (dV)/(dt) = alphaPA_B - EA_L + G_i - G_o + S_i - S_o `
where ` (dV)/(dt) ` is the change in lake volume with time, `P` is the amount of precipitation falling on the drainage basin that feeds the lake, `A_B` is the area of the lake basin, `alpha` is a runoff coefficient describing the percentage of the precipitation that makes its way to the lake without being intercepted by plants or soaking into the soil to enter the groundwater system, `E` is the rate of evaporation of lake water, `A_L` is the lake surface area, `G_i` is the amount of groundwater discharging into the lake, `G_o` is the amount of water leaving the lake to enter the groundwater system, `S_i` is the amount of water spilling into the lake from the next lake higher in the chain, and `S_o` is the amount of water spilling out of the lake basin to the next lake lower down the chain (Mifflin and Wheat, 1979; Phillips and others, 1992).
The low permeability of the the lake sediments in each basin in the chain prevents much groundwater exchange with the lake, so we can ignore `G_i` and `G_o`. For a steady state lake that is neither gaining nor losing water by spillover, ` (dV)/(dt) `, `S_i`, and `S_o` all equal zero, so the equation above becomes:
` 0 = alphaPA_B - EA_L ` or
` alphaPA_B = EA_L `.
Let us think about what this means. Since the area of the basin is a fixed parameter, any increase in the amount of runoff will create a larger lake given the same evaporation rate. Likewise, a decline in evaporation rate would result in a larger lake if runoff were to remain constant.
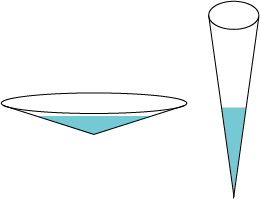
![[creative commons]](/images/creativecommons_16.png)
References
Gilbert, G.K., 1875, Report on the geology of portions of Nevada, Utah, California, and Arizona examined in the years 1871-1872: U.S. Geographical and Geological Surveys West of the 100th Meridian, v. 3, part 1.
Kutzbach, J.E., "Model simulations of the climatic patterns during the deglaciation of North America," in Ruddiman, W.F., and Wright, H.E., Jr., eds., North America and adjacent oceans during the last deglaciation: Boulder, Colorado, Geological Society of America, The Geology of North America, v. K-3, p. 425–446.
Mifflin, M.D., and Wheat, M.M., 1979, "Pluvial lakes and estimated pluvial climates of Nevada," Nevada Bureau of Mines and Geology Bulletin, n. 94, 57 p.
Phillips, F.M., Campbell, A.R., Kruger, C., Johnson, P., Roberts, R., and Keyes, E., 1992, "A reconstruction of the water balance in western United States lake basins to climatic change," New Mexico Water Resources Research Institute Technical Completion Report, n. 269, v. 1.
Phillips, F.M., 2008, "Geological and hydrological history of the paleo-Owens River drainage since the late Miocene," in Reheis, M.C., Hershler, R., and Miller, D.M., eds., Late Cenozoic Drainage History of the Southwestern Great Basin and Lower Colorado River Region: Geologic and Biotic Perspectives: Geological Society of America Special Paper 439, p. 115–150.
Reheis, M.C., Adams, K.D., Oviatt, C.G., and Bacon, S.N., 2014, "Pluvial lakes in the Great Basin of the western United States — a view from the outcrop," Quaternary Science Reviews, v. 97, p. 33–57.
Smith, G.I., and Bischoff, J.L., 1997, "Core OL-92 from Owens Lake: Project rationale, geologic setting, drilling procedures, and summary," in Smith, G.I., and Bischoff, J.L., eds., An 800,000-Year Paleoclimatic Record from Core OL-92, Owens Lake, Southeast California: Boulder, Colorado, Geological Society of America Special Paper 317, p. 1–8.
Smith, G.I., Bischoff, J.L., and Bradbury, J.P., 1997, "Synthesis of the paleoclimatic record from Owens Lake core OL-92," in Smith, G.I., and Bischoff, J.L., eds., An 800,000-Year Paleoclimatic Record from Core OL-92, Owens Lake, Southeast California: Boulder, Colorado, Geological Society of America Special Paper 317, p. 143–160.
Smith, G.I., and Street-Perrot, F.A., 1983, "Pluvial Lakes in the Western United States," in Wright, H.E., Jr., ed., Late Quaternary Environments of the United States: Minneapolis, University of Minnesota Press, p. 190-211.