For the Instructor
These student materials complement the Renewable Energy and Environmental Sustainability Instructor Materials. If you would like your students to have access to the student materials, we suggest you either point them at the Student Version which omits the framing pages with information designed for faculty (and this box). Or you can download these pages in several formats that you can include in your course website or local Learning Managment System. Learn more about using, modifying, and sharing InTeGrate teaching materials.Student Reading: Creating Electricity from Light
Learning Goals: Students will be able to:
- Recount the fundamental principles of electricity to include the concepts of charge, current, voltage, resistance, and conduction.
- Contrast parallel and series circuits.
- Articulate how the photoelectric effect is harnessed in photovoltaic (PV) cells to create the flow of electrons.
- Distinguish between concentrated and dispersed solar-electric production and note the advantages and disadvantages of each.
- Evaluate the important parameters used for location of solar system (latitude, slope, aspect, shading, and cloudiness).
- Determine economic cost and environmental impacts of PV technology.
- Differentiate between the different approaches to using concentrated solar production to make electricity.
- Distinguish between grid-tied and stand-alone photovoltaic systems and the advantages and disadvantages of each.
- List and explain the limitations of using sunlight to produce electricity.
Electricity from Sunlight
Solar thermal generators
A traditional steam engine uses heat from burning a fossil fuel to boil water. The resulting pressurized steam drives the engine (or turbine) which can then turn an electric generator. Indeed, this is how commercial electricity has been made since Edison's first power plant went online in 1882. Concentrated solar thermal (CST) generators use sunlight instead of fossil fuels to provide the heat. They rely upon a system of mirrors to concentrate the sunlight. These plants can produce significant amounts of electricity. Augustin Mouchot, a French high school math teacher, pioneered this technology in the 1860s. Captain John Ericsson is most famous for designing the revolutionary iron warship called the USS Monitor. The Monitor played a pivotal role in the US Civil War and was the prototype of battleships for the next eighty years. In his later years he turned his attention to solar energy and designed a heat engine run by the sun. It was designed to pump water from wells. Ericsson's design coupled a parabolic lens with a Sterling engine — essentially the same elements found in modern CST systems. While neither Mouchot's nor Ericsson's devices produced electricity, they did demonstrate the feasibility of using solar thermal energy to make mechanical energy, which could then be used to turn a generator. Watch this YouTube video: Replica of Ericsson's sun engine
The largest CST system currently operating consists of nine separate plants tied together and is rated at 354 megawatts. It is located in the United States in the Mojave Desert. For comparison, nuclear power plants in the United States have generating capacities that range from 478 megawatts to 3,937 megawatts. Typical coal-fired power plant capacity is 667 megawatts.
Over one hundred solar thermal electric plants are in operation worldwide, and a similar number are under construction. There are two main designs. The most common one uses parabolic solar troughs to heat tubes containing a liquid. The other, less common approach uses a field of mirrors to focus sunlight on a collector in a tower. In addition to solar troughs and tower systems, two other approaches are used. The Stirling-Dish system uses a large parabolic dish (think of a large satellite dish) to focus light on a Stirling steam engine held in place by an arm from the dish. Some trough systems use prisms or Fresnel lenses to help concentrate light.
All of the CST systems use light to heat a fluid. The heated fluid is used to transfer thermal energy to water to make steam and turn an electric generator. The heated fluids are typically liquid salts that have a very high heat capacity. Sodium nitrate, calcium nitrate, and potassium nitrate are often used. Using molten salts rather than water to collect the heat has advantages. The salts have a much higher heat capacity than water, so they can store more heat. That heat can be used to create steam even after the sun has set. These plants will cycle the molten salts between 288 Co and 566 Co.
Some CST plants are hybrid systems. When the heat stored in the molten salts is exhausted later in the night, or if there is a cloudy day, natural gas is burned make the steam and to maintain the production of electricity.
The best CST systems are about 30% efficient in converting the energy in sunlight to electricity. This is better than the 20% efficiency typical of photovoltaic (PV) systems discussed below. However, the CST systems are complex, with many moving parts and high-pressure fluids. They work best when the reflectors move to track the daily transit of the sun (heliostats), and that requires a complex system of small motors controlled by computers. View a short video on Energy 101: Concentrating Solar Power from Energy.gov Office of Energy Efficiency & Renewable Energy.
Another large-scale way to turn solar energy into electricity is with a solar updraft tower system. These consist of very large greenhouses that heat air at ground level. The hot air escapes through a very tall chimney or tower. A wind turbine in the tower is turned by the up-rushing air. So sunlight is converted to thermal energy, and that is converted to mechanical energy, which is then converted to electricity. Only a few of the updraft plants have been built. They are much less expensive to construct than a solar steam plant, but do not produce much electricity.
All CST installations require large expanses of land in sunny areas. So they are usually placed in deserts or semi-deserts. Such installations are typically far from urban centers where the electricity is needed. This means construction of high voltage power lines to carry the electricity to the grid. Each station also destroys or significantly alters large areas of the natural desert ecosystem.
A mechanical device that rotates an electrical generator is at the heart of all CST systems. The two most common devices are turbines and Stirling engines. Watch these YouTube videos: How a Stirling engine works and How a steam turbine works. Note that the steam turbine in this video assumes the steam is created by a fossil fuel, which would not be the case for a CST plant. Yet some hybrid CST installations do use natural gas as a backup fuel for nighttime or cloudy days.
Thermal electric generators
Another way to turn thermal energy from sunlight (or any source) into electricity is with a thermoelectric generator. These work by using the thermoelectric effect, first discovered in the early 19th century. The simplest of these is based upon a loop of wires consisting of two different metals. When heated, electrons will flow around the circuit, as long as one portion of the circuit is cooler than the other. More sophisticated devices use sandwiches of semiconductors, with one N-doped layer and one P-doped layer. The N-doped layer is a source of electrons and the P-doped layer is a sink for the electrons. Applying heat will cause the electrons to flow through a circuit. The amount of useful energy produced depends on the temperature difference in the system. On a small scale, thermoelectric generators are used to regulate equipment, essentially like a thermostat. But to make significant amounts of electricity there needs to be a large temperature difference. One can buy such generators to place upon a hot wood-burning stove. The electricity produced can turn a small fan or charge 1.5 volt batteries. Devices such as the Seebeck module are also used in automobiles to get electricity from the hot engine. Power plants can also use their waste heat to make additional electricity with thermal electric generators.
Thermoelectric generator ( 6kB Jun11 14)
The thermoelectric effect can also be used for modest refrigeration. By passing electricity through the device, it causes cooling. The devices do not provide much cooling for the energy consumed, but they are a good way to maintain temperature in a small cooler of food that one is keeping in a car. These run on the electricity produced by the car's alternator. These are commercially available and marketed to campers and boaters.
Photovoltaics
All of the previous methods of turning sunlight into electricity involved the intermediate step of first changing that light into heat. However, in 1839 Edmond Becquerel of France discovered that light can be turned directly into electricity. In 1905, Albert Einstein published his Nobel Prize-winning work that explained Becquerel's photoelectric effect and the general properties of light. In 1954 the Bell Laboratories produced the first practical photovoltaic system based upon the photoelectric effect. Yet it was not until later that decade that it got its first real use. In 1957 the Soviet Union began the "Space Race" with the launch of its Sputnik satellite. The United States followed the next year with its own Echo satellite. These early space probes ran on batteries, and when the batteries went dead, so did the very expensive satellite. Photovoltaic systems proved ideal for keeping the batteries charged. In orbit high above Earth, the satellites had plenty of sunshine. Earth's atmosphere reduces the amount of sunlight getting to the surface by about half on a clear day, and clouds may reduce it another 90%. Satellites in space are above all that. The need to power the fleet of satellites led to intense research in photovoltaic cells.
Although photovoltaics found their way into some small devices back on Earth, such as watches during the 1960s and early 1970s, they were not given serious consideration for making significant amounts of electricity until 1974. That year a war in the Middle East prompted a shortage of oil in the United States and Europe, called the energy crisis. The price of gasoline and heating oil doubled, and long lines of cars formed at gas stations. This caught the attention of the public, and it stimulated research on alternative sources of homegrown energy. View excellent short videos on Solar PV from Energy.gov.
How PV works — PV cells use the photoelectric effect. In the simplest form, they consist of thin wafers of a semiconductor (silicon) in a sandwich. One layer is doped with phosphorus (N for negative) and the other is doped with boron (P for positive). The term "doped" means that something was added to the silicon wafer. The N- and P-doped layers are separated by a tiny space called the P-N junction. When sunlight hits the PV cell, it drives electrons from the N-layer to the P-layer. By connecting the two layers with a wire, a circuit is created as the electrons flow back to the P-layer. That flow of electrons can be then used to do work. When the light goes away, so does the electron flow.
Silicon PV panels found on rooftops today convert roughly between 15 and 22% of the energy in light into electrical energy. Efficiency varies depending on the type of silicon technology, the purity of the materials used to make the solar cells, and the quality of fabrication used in manufacturing the PV panel. Generally speaking, amorphous and polycrystalline silicon cells have lower efficiency, while high-quality monocrystalline panels perform better. High-efficiency PV modules cost more, but use less space to produce the same amount of electricity as less-expensive units.
Advanced multi-junction PV cells use stacks of different kinds of doped silicon layers to maximize the capture of the different wavelengths of sunlight. Typical configurations include a triple junction to absorb the red, green, and blue portions of the solar spectrum. In the laboratory, multi-junction PV cells have demonstrated efficiencies as high as 46%. However, the high cost of multi-junction solar cells has generally limited their use to special applications, for example in space missions such as the Mars Rover.
No matter how big the PV cell, the difference in electrical potential between the two layers (the N-P junction) is only about 0.6 volts. A bigger cell will provide more current (more electron flow) but no more voltage. To get more voltage, multiple PV cells are connected in series. So if one needed 3 volts for a particular application, 5 PV cells could be strung together in series (5 x 0.6 V = 3.0 V). Most applications, and certainly those that are designed to produce significant amounts of electricity, will use many PV cells connected in series. A small group of such connected cells is called a module, and modules are often connected together to form an array.
Consider the 215 watt-panel shown to the far right. How much voltage does it produce? There are 12 rows of 6 PV cells each, or 72 cells. 72 x 0.6 V= 43.2 V. Ten such modules are often wired together in series, and that produces 432 volts! For comparison, a flashlight typically uses 3 volts, a car battery is rated at 12 volts, and household current in the United States is 120 volts.
There are two different approaches to using PV, stand-alone and grid-tied systems. Stand-alone systems consist of PV modules connected to storage batteries. They are self-contained systems that produce direct current (DC) and store that energy in batteries. A voltage regulator is usually installed to protect the batteries from the damage of overcharging. These systems are often found on sailboats or in cabins that are distant from the electrical grid. Most lights and electronics on boats require 12 volts, DC, so they are a good match for the stand-alone approach. Cabins might have larger appliances, such as a washing machine, television, or vacuum cleaner. These appliances are normally sold in the United States designed to run on AC power. However, DC versions are available, but usually at a higher price. A power inverter can change the DC electricity to AC, but at a cost of about half the energy being lost to heat. Stand-alone systems require a large investment in storage batteries and the batteries must be maintained regularly and replaced about every five years. Finally, any capacity to produce electricity beyond keeping the batteries charged is wasted. It is impossible to share that extra capacity with someone who needs it.
Grid-tied systems consist of PV arrays and power inverters connected to the AC power delivery system that serves the entire community (and nation in the United States). The inverter is required to change the DC electricity produced by the PV array to the AC current distributed by the grid. A good modern inverter is 95% efficient, i.e., only 5% of the DC electricity energy is lost as it is converted to AC. A grid-tied system offers several advantages over the stand-alone approach. Rather than investing in batteries, the grid-tied system uses the grid like a battery. If more energy is required by a household with a grid-tied system than is being produced at any one time, then the grid provides the electricity to make up the difference. At night or on a very cloudy day, the grid powers the house. When the PV system is producing more energy than is being used by the house, the excess is exported to the grid. Grid-tied systems connect to the grid through special meters that record the direction as well as the amount of electricity flowing. Such net-metering devices keep track of how much net electricity is used or produced. The electric utility company will charge for the net amount of electricity used each month. If the PV system produces more than the house used that month, then the utility will provide a credit to the account. At the end of a year, most utilities will pay for any extra produced by the PV system. However, although electric utilities sell electricity at the retail rate (national average of 11 cents per kWh), some might only credit the PV system owner for electricity generated by the solar system at the wholesale rate (national average of 4 cents per kWh). Net-metering policies differ depending on state laws and on regulatory decisions that are made in public utility rate cases.
Another advantage of grid-tied systems is that owners of such units can recoup some of their investment by selling the RECs (Renewable Energy Credits) that they produce. This is different from simply selling back extra production to the utility. The concept is that every megawatt of clean renewable energy produced by the PV system displaces a megawatt of non-renewable energy that would otherwise be made by coal, gas, or nuclear plants. Many states have mandated or set voluntary goals for their utilities to get a specified percentage of the electricity they sell from renewable sources. In response, some utilities invest in renewable energy production by building wind- or solar-powered installations.
The utilities can also satisfy the renewable energy goal by purchasing RECs from industrial-scale operations like wind or solar farms operated by others, or from individual homeowners. Understand that purchasing the RECs involves no actual buying of electricity. The REC is simply paying someone else for the fact they created that megawatt of renewable energy. Homeowners can pool their RECs to be sold on the market by brokers who take a commission for their work. The price of RECs is highly variable, ranging from about $25–$250 per megawatt. Producers with long-term contracts dating to about 2008 can expect the higher price for their RECs. If more states require utilities to use renewable energy, and if the states also raise the amount that must be used by the utility, REC prices will rise accordingly. As of 2017, twenty-nine states, Washington, D.C., and three US territories had mandated Renewable Portfolio Standards (RPS), while eight states and one territory had unenforceable goals. California has the strongest RPS requirements, with mandated levels of renewable energy comprising 20% by the end of 2013, 25% by 2016, 30% by 2020, and 50% by 2030 ( RPS for California). Since California is such a large state, this should help drive a strong REC market price. US maps showing aspects of solar and renewable energy policy
Distributed vs Large-Scale Energy Production
The familiar model of electricity production is to build large centralized power plants. A problem with this approach is that the energy is often produced some distance from where it is needed, and expensive high voltage wires must be installed to deliver the energy. Along the way, a portion of that energy is lost as heat. PV systems naturally lend themselves to the alternative model called distributed energy production or distributed energy resources. The distributed approach links together many small energy producers and storage systems (batteries). This puts the energy production closer to where it is needed and allows coordination of different types of generators that complement each other. For example, PV, wind, solar thermal, and fuel cells and batteries can all be linked. If the sun is not shining or the wind does not blow, batteries and fuel cells can satisfy demand.
Another advantage of distributed production for PV systems is that sunny rooftops can be put to good use as places to generate clean electricity. An added benefit is the shading provided by the PV collectors. This reduces the summertime heat load on the roof, keeping the building cooler below. Does it really make sense to spread PV collectors over desert habitat or farm land when so much empty roof space is available much closer to where the energy is needed?
Types of PV Cells in Use
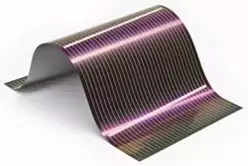
Polycrystalline cells are made of bits or small chunks of the silica wafers, arranged in a random pattern. They are cheaper to produce, but less efficient than monocrystalline cells. They appear blue, as they do reflect some of that portion of the spectrum. The random orientation means they are better at working under diffuse light conditions, such as cloudy or hazy conditions. Efficiency of 10–15% is typical.
Thin film cells are created with a liquid that is placed between two layers of glass. They are relatively new on the market, cheaper to make, but less efficient than poly or mono crystalline versions. A big advantage of thin film cells is the flexibility of the panels constructed from them. They are excellent for portable applications such as boating and camping.
Solar in Transportation
The first practical application for PV solar was in transportation, powering satellites. The PV energy was not for moving the spacecraft, but for powering their instruments. PV has been adapted for recreational sailboats that make long voyages and need electricity for lights, communication, ventilation, instruments, refrigeration, and self-steering.
NASA has tested a solar-powered aircraft called the Helios Prototype. There is an effort to fly such a plane (the Solar Impulse) nonstop around the world.
Limitations of Solar Energy
If 0.1% of Earth's surface was covered with PV panels, it would meet all of the energy needs of the world! That is about the area of Spain, or between that of California and Texas.
(How much land for solar?) But it is more complicated than that.
Solar energy requires sunshine, and sunshine is not distributed evenly around the world in either time or space. Recall that Earth is tilted on its axis (23.4o). This has a profound effect on how Earth is illuminated. If the axis was not tilted, everywhere on Earth would experience the same pattern of day and night, twelve hours of darkness followed by twelve hours of sunshine every single day of the year. But Earth is tilted. For half of the annual journey around the sun, the Northern Hemisphere is tilted toward our home star and the Southern Hemisphere is tilted away from it. This reverses for the second half of the year. When a hemisphere is tilted toward the sun, it gets more sunlight for two reasons. First, the sun appears higher in the sky, so the sunlight is more direct (closer to perpendicular to Earth's surface) and the length of the days (photoperiod) is longer. The longest day of the year in that hemisphere is called the summer solstice. In the other hemisphere, it would be the shortest day of the year, or winter solstice. Twice each year there is a day (the spring and fall equinox) where for the entire planet there are twelve hours of light and twelve hours of darkness.
Near the Equator there is little difference in day length of solar illumination over the course of the year, days and nights remain about 12 hours long each. The Arctic and Antarctic Circles (66.6o) indicate where there will be one full day of sunlight on the summer solstice and one full day of darkness on the winter solstices. Latitudes above these circles experiences multiples days of full sunlight and full darkness. Solar energy is available at about the same rate all year long in the tropics. At higher latitudes the energy is concentrated in the months around the summer solstice. Yet even at higher temperate latitudes, the low sun can shine brightly through cold, clear winter skies and provide some useful energy for a few hours a day.
In addition to the length of a day, calculations of potential energy from the sun must consider the angle at which the light intersects the surface of Earth. Around the equator the sun crosses the sky nearly directly overhead each day during the year. The Tropics of Cancer (Northern Hemisphere) and Capricorn (Southern Hemisphere) mark places where the sun passes directly overhead one day each year. At higher latitudes the sun's rays intercept the level surface at an angle. So the same amount of sunlight that strikes one square meter of land near the equator will be spread out over twice that area in the Arctic.
There are other limitations on solar energy. For collectors to be efficient they must be located well above or away from the shade of trees and tall buildings. Clouds, fog, smog and dust all reduce the solar energy reaching a collector.
Collectors work best when oriented perpendicular to the sunlight, and since the solar angle changes over the course of a day or season, one either has to use one fixed optimal setting or have a way of adjusting the device on a regular basis. The best tracking systems have small electric motors to constantly adjust the collector (or mirror for CST) for the best angle. These heliostats, like sunflowers, follow the sun all day. Such devices are expensive and require frequent maintenance.
Even in the sunniest deserts of the world, the sun will disappear at night, and so does the capture of energy until the next morning. As with wind energy, there are times solar generators will be off-line. As such they should be tied to grids that include short-term energy storage devices.
Many communities have restrictive laws limiting the installation of solar devices on houses and adjacent property. Even if there are no such restrictions, once does need to acquire a green aesthetic where beauty is found in the functionality of PV panels.
Which locations lead in using solar energy to make electricity?
Solar Power Rocks is a lobbying group that promotes policy changes to facilitate solar energy in the United States. They rank the fifty states and Washington, D.C. See Solar Policy by State. As of 2017, Massachusetts ranks highest and Mississippi the lowest. Note that the rankings mostly correspond to the payback period for installing a solar system on a house. Putting a 5 kW system on a house in Massachusetts will pay for itself in savings on the utility bill in just four years, while it will take sixteen years to recoup the cost for the same system in Mississippi.
As of 2017, the top solar-power producing states are, in order: California, Arizona, Massachusetts, New Jersey, and New York (Ranking of states for production of solar energy). Note that the first two on the list are large and sunny. The next three are smaller and not as sunny, so why do you think they achieved such high ranks? Think about the role of policy.
Solar and wind energy are now the fastest-growing and least-expensive ways to produce electricity. As of 2017, solar energy accounts for 1.8% of the electricity made worldwide. Leading the way, in order, are: China, Japan, Germany, United States, and Italy. On a per capita basis, Germany produces 511 watts per person, Japan 336 watts and Italy 332 watts. See: Solar Production by Country
Collecting your thoughts: Systems thinking and reflection
You have just learned about the history of using energy from the sun to create electricity. Take a few moments to consider how this all fits together. You also have already learned about how wind energy is used to create electricity. In what ways are are wind and photovoltaic/CST technologies similar, and in what ways are they different? Which technology is best suited for the area where you live, and why?
Most PV systems are grid-tied. Think of the nation's electrical grid as a system that has to deliver constant current and voltage on demand. What are some events that might cause PV systems to destabilize that system? What are some ways of preventing that instability?
What would be the pros and cons of using photovoltaics on your college campus?
- How PV Works, from Energy.gov's Office of Energy Efficiency & Renewable Energy
- Annual solar cycle graph from physicalgeography.net
- Back to the Science@NASA story "The Edge of Sunshine"
- NREL Solar Resource maps — find the annual average energy for where you live.
- Planning a home PV system, from Energy.gov
- Experiment to do electrolysis using solar energy from the New Mexico Solar Energy Association (p. 136)
- Article about the Basics of PV from Energy.gov's Office of Energy Efficiency & Renewable Energy